Enhancing the infrastructure and operational resilience of power systems against wildfires
It is widely recognized nowadays that wildfires are connected to extreme weather events since their ignition and development are caused by extreme temperatures and dry weather conditions. In fact, the frequency and intensity of wildfires are expected to increase in the coming years due to climate change and the resulting increase in the temperature of the environment. Recent work by the CIGRE Working Group C4.47 “Power System Resilience” [1] indeed indicates a consensus from electricity utilities around the globe on the increasing need of assessing and enhancing power system resilience against extreme events, including wildfires.
By Mathaios Panteli, Rodrigo Moreno, Dimitris Trakas, Magnus Jamieson, Pierluigi Mancarella, Goran Strbac and Nikos Hatziargyriou
The majority of wildfires are caused by human activity, e.g. due to arson, negligence or accidents. Among the latter, powerlines play a predominant role. In particular, powerline-involved wildfires typically occur under worse fire conditions (high winds, low moisture/humidity) resulting in rapid fire growth. As a result powerline-involved fires are typically larger and faster spreading than general wildfires. Wind plays a major role in causing powerline-involved wildfires by either directly interacting with the powerlines or indirectly causing foreign objects to interact with them. One direct effect is conductor clashing where two conductors make contact, allowing current to flow between them, heating the powerlines to the point of melting. Heated molten droplets or solid particles of conductor material may be ejected and depending on the material (e.g., aluminium), they may exhibit flaming combustion. When falling onto the ground they may ignite fuels under favourable conditions. Wind can indirectly cause wildfires by causing trees or large branches falling and breaking powerlines. Broken conductors falling to the ground can ignite vegetation as a consequence of electrical discharge or come into contact with other conductive material (telecom lines, support wires, etc.), resulting in conductor clashing. Fallen trees can also provide a path for electrical current, heating the vegetation and igniting it producing spreading flames.
High intensity wildfires can also provoke damage to powerlines by producing flashovers due to increased electric conductivity of the hot combustion products or due to deposition of particles on suspension insulators. Flames near conductors can provide a conductive path through the ionized gas species present in the flame, promoting flashover. Heat from wildfires can increase the temperature of powerlines above permissible limits causing the conductors to sag which combined with wind can cause conductor clashing faults during fires. Wooden poles of power lines and transformers can burn during fires, severely damaging power distribution networks and aluminium conductors may melt due to the low melting point of aluminium (~640oC). Fig. 1 shows damaged poles from the 2007 wildfires in Greece.
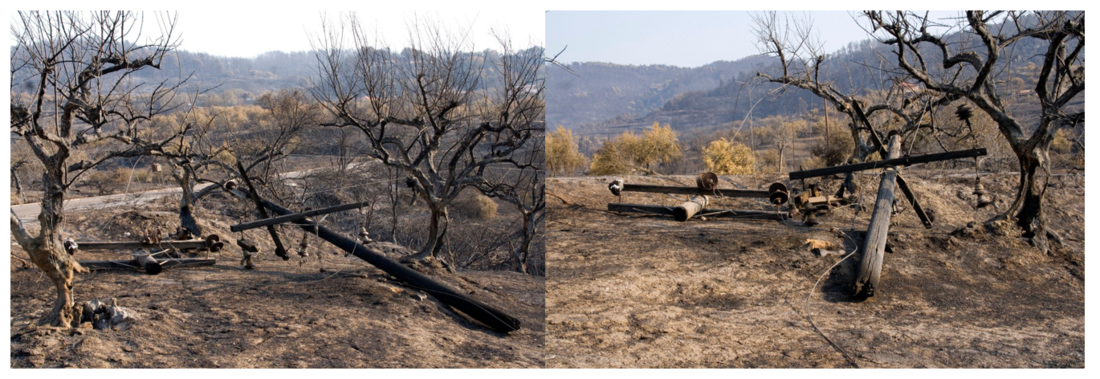
Figure 1 - Damaged poles from the 2007 forest fires in Greece
(Source: Courtesy of Public Power Corporation, Greece)
The majority of electric utilities around the world have recognized the need for taking measures to enhance the resilience of the power system. The relevant efforts aim to adapt the network’s structure and operation to minimize the consequences of future extreme events. This can be achieved by enhancing the system before and during the development of the extreme event and/or by optimizing its performance after the event. This can be distinguished in infrastructure hardening and operational/smart measures. Infrastructure hardening measures aim to make the system assets less vulnerable to extreme events while operational measures include mainly intelligent control that aims to face the events while they progress by adapting the system’s operation. They aim to let the system “bend” without “breaking.”
This paper provides an overview of measures, both related to infrastructure resilience and operational measures taken by Utilities operating in parts of the world that have suffered large wildfires in the last years. These include California, Latin America, Australia, and Greece. Emphasis is given on the exploitation of distributed generation and microgrids in enhancing system resilience, including real world examples.
Asset and system vulnerability assessment against wildfires
During periods of high risk of wildfires, it is critical to predict, as accurately as possible, the effect of a wildfire on the power system assets. Different models, for example based on regression models, have been developed for forecasting the probability of failures of high-voltage transmission and distribution lines when exposed to wildfire conditions. These conditions include both intense smoke and high temperatures which can result in the operational de-rating of a line and its temporal availability and operational status based on the high uncertainty related to the wildfire trajectory across the system. These line outages can be transient and/or temporary, both introducing though operational challenges to the system operator. System operators often need to permanently switch off a line that is experiencing frequent and high numbers of transient outages and reclosures to reduce the operational uncertainty.
This predicted asset behaviour is usually fed into advanced stochastic models for quantifying the spatial and temporal behaviour of the system as a whole. Various metrics are used in such analyses, including number of assets tripped and amount, duration and frequency of load curtailment. Recent reports and guidelines advise strongly in favour of not using only the average/expected values of such network performance metrics, but also their conditional values which clearly focus on and capture the “tail” risks and hence high-impact, low-probability events. Risk metrics, such as Value at Risk and Conditional Value at Risk, can be used in this context.
Restoration and recovery, as key elements of resilience and setting it apart from other concepts (such as reliability and security), are of growing concern among system operators and decision-making bodies. The spatial and temporal modelling and quantification of the system topology and load curtailment due to the wildfire enables the quantification of the desired restoration times which will drive the prioritisation of the recovery strategies (e.g., dispatch of repair crews or mobile energy resources). This holistic analysis is then used to generate the performance curve of the event during and after the event, which supports the longer term adaptation and mitigation strategies for the resilience enhancement of the system.
Resilience enhancement against wildfires
Infrastructure resilience
Protecting power systems against natural hazards requires addressing the so-called resilience trilemma (Fig. 2), that is, the need for balancing the portfolio solution among several options to make the system more robust (stronger), more redundant (bigger) and more flexible and responsive (smarter) in a resilient and cost-efficient manner. Fig. 2 shows examples of such sets of investment decisions to enhance system resilience, which would be complementary within resilience enhancement portfolios in order to achieve effective trade-offs between cost and resilience enhancements.
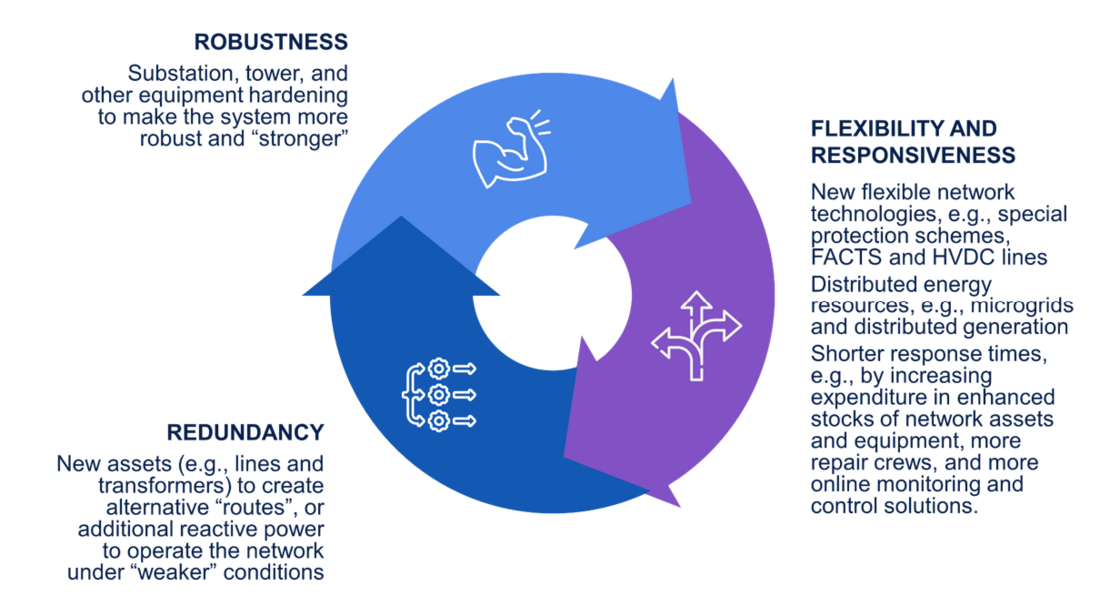
Figure 2 - The resilience enhancement trilemma
Examples of hardening decisions include undergrounding lines to deal with high wind speeds, upgrading poles with stronger, more robust materials, building substation defences to deal with flooding events, and anchoring substation equipment to better deal with earthquakes. In case of wildfire hazards, we can consider the following list of expansion and hardening measures:
- Undergrounding lines as an extreme measure of hardening.
- Upgrade poles/towers and conductors to harden the powerline, for example, increasing the heights of towers and using covered conductors.
- Increase the width of the right-of-ways, and improve vegetation and fuel management to minimise both the risk of ignition and the spread of a wildfire approaching the power network.
- Install new lines and distributed energy resources (DERs) such as generation and storage facilities (which can be mobile), as an alternative way to provide electricity in case the current supplying network corridors are at risk.
Besides expansion and hardening measures, resilience against wildfires is boosted by the application of various non-wires, smart technologies at various stages discussed in Section 3.b.
Asset management technologies and inspection
A very large amount of images collected by helicopters and drones in potential high fire risk areas, can be used to detect broken conductor strands or growing tree branches that might contact electrical lines. Application of camera image processing technologies, such as using machine learning algorithms, can be used for the automated examination of assets and identification of pre-failure conditions before a failure and, possibly, ignition occurs. So far, image analysis is demonstrating a higher rate of circuit issue detection than human inspection. Further emerging technologies, such as infrared and corona detection, can proactively identify asset defects that may not be visible to the human eye (e.g., hotspots on a wire).
Situational Awareness and Forecasting
Advanced operational models for wildfire risk based on weather and fuel moisture data, mountaintop camera network integration, weather station data, wind deviations based on measurements across the service territory, and fire simulation analytics for all reported triggering events have been developed. Wide-area situational awareness (WASA) and transmission visualization systems have been developed based on PMUs that provide electrical data at high rates to system operators at control centers for situational awareness of the dynamic behavior of the grid. Several wildfire management applications are being developed for the WASA system, including multi-layered GIS map-based visualization software that will show accurate fault location, plus point-on wave event and weather conditions (wind, fire, and lightning). This helps the operator to quickly dispatch personnel, conduct event analyses and restoration, and manage catastrophic events. As an example, Fig. 3 shows a snapshot of the advanced situation awareness tool managed by the Ministry of Energy in Chile, a territorial viewer that allows permanent monitoring of the main threats affecting the energy sector, including wildfire threats.
Advanced protection
The large majority of wildfire ignition caused by aerial powerlines involve an electrical fault. Advanced protection systems include increased sensitivity and speed of line protection. These include Sensitive Relay Protection (SRP) settings that are engaged by distribution operators at times of high fire risk. The setpoints are set as sensitively as possible without tripping for normal load conditions in order to clear a fault very fast. Another layer of risk reduction is Sensitive Ground Fault (SGF) protection to detect distribution ground faults caused by energized conductors on the ground that cause very little fault current that cannot be detected by standard ground overcurrent protection settings. Advanced protection programs also include Falling Conductor Protection (FCP) systems that detect a broken energized conductor and de-energize it before it strikes the ground, eliminating the risk of fire ignition or public exposure to live conductors. This technology uses synchrophasor-based distribution circuit monitoring.
Operational resilience
Simply making the power system smarter is not enough to improve its resilience against wildfires and extreme events in general. It should also operate in a smart way, making effective use of the installed DERs and network technologies [2], mentioned in the previous section. Concerning wildfires, these system assets can be utilized to minimize load shedding when (Public Safety Power Shutoffs) PSPS are applied to prevent a fire ignition from electric grid faults during periods of high winds and low humidity or when a progressing wildfire threatens the smooth operation of the power system.
During PSPS events, power components are selectively de-energized to reduce the risk of wildfire ignition, leading to unavoidable load curtailment that can last from hours to days. Therefore, while PSPS are the only short-term measure to eliminate the risk of a fire ignition, they have significant economic and social implications due to the power outages. PSPS strategy should be a trade off between wildfire risk and power outage disruptions. DERs and network technologies can play a key role in minimizing power outages when power components are turned off. DERs can meet the demand locally without relying on long powerlines that might be a fire ignition threat. In addition to the already installed DERs, mobile DERs can be connected to the network to reduce load shedding. Since PSPS are applied during days with high solar irradiation level, when the risk of a fire ignition is high, the use of energy storage systems (ESS) is critical for storing the surplus energy produced by the PVs during the day and use it at night. Network reconfiguration can also reduce the use of system components located or passing through wooded areas and therefore have a high risk of initiating a fire. Furthermore, network technologies, such as FACTS can be utilized to eliminate congestions due to the reduced network capacity when lines are de-energized.
In case of a progressing wildfire, the DERs should be scheduled and dispatched taking into account the system components that are threatened by the wildfire. In case of damaged components, parts of the system might be isolated or lines might be congested. In both cases, already installed or mobile DERs, in combination with an effective network reconfiguration, can minimize load shedding, either by forming self-sufficient microgrids or by meeting the demand locally, reducing the flow in lines. The use of a situational awareness system, in conjunction with a geographic information system (GIS), is vital to detect the wildfire and estimate its progression in order to assess the spatiotemporal impact on these components. Having assessed the vulnerable components and the impact on them, the preemptive operation strategy of the system is developed, taking into account the stochastic nature of renewables. It is highlighted that the impact on the DERs should be also taken into account. For instance, the effect on the PV generation due to reduced sunlight as a result of the wildfire smoke should not be neglected.
In addition to proactive measures, corrective measures during system restoration, after the fire has been extinguished, are of high importance. These measures include the rapid identification of the damaged components and the development of a restoration plan. Restoration plan should consist of a repair sequence of the damaged components that accelerates the reconnection of the customers, as well as DER utilization and network reconfiguration that takes into account the progress of repairs.
Real world examples
There have been several examples across the world of different measures to improve power system resilience to wildfires.
The power grid in California must frequently disconnect large sections during windy and dry weather to prevent them from causing wildfires. PSPS while generally effective, may leave customers disconnected for days and at significant cost. However, such costs are overall justified looking at the potential impact of wildfires. A most significant case is, for instance, the “Camp Fire” of Nov. 8, 2018, in the northeast of the state, caused by the failure of a hook carrying a conductor, which led to 85 fatalities and Pacific Gas & Electric Company (PG&E) to file bankruptcy. In order to minimise customer impact as well as provide system resilience, new solutions based on microgrids are thus now also being developed throughout the state which can operate independently of the main grid when it is disconnected.
DERs are being increasingly deployed for system restoration purposes too. For example, when severe wildfires in Greece caused supply disruption to almost 100,000 customers between Aug. 24 and Sept. 7, 2007, mobile diesel generators provided tremendous support to recovery of several rural areas. Similarly, widespread wildfires in 2-8th August 2021 caused outages to almost 50,000 customers spread across different parts of the country, and some 5 MW of diesel generators supported system recovery, especially to power emergency services, eventually managing to restore electricity in all areas within only 10 days. Fig. 4 shows mobile diesel generators used in Greece during system restoration after the wildfire in 2007.
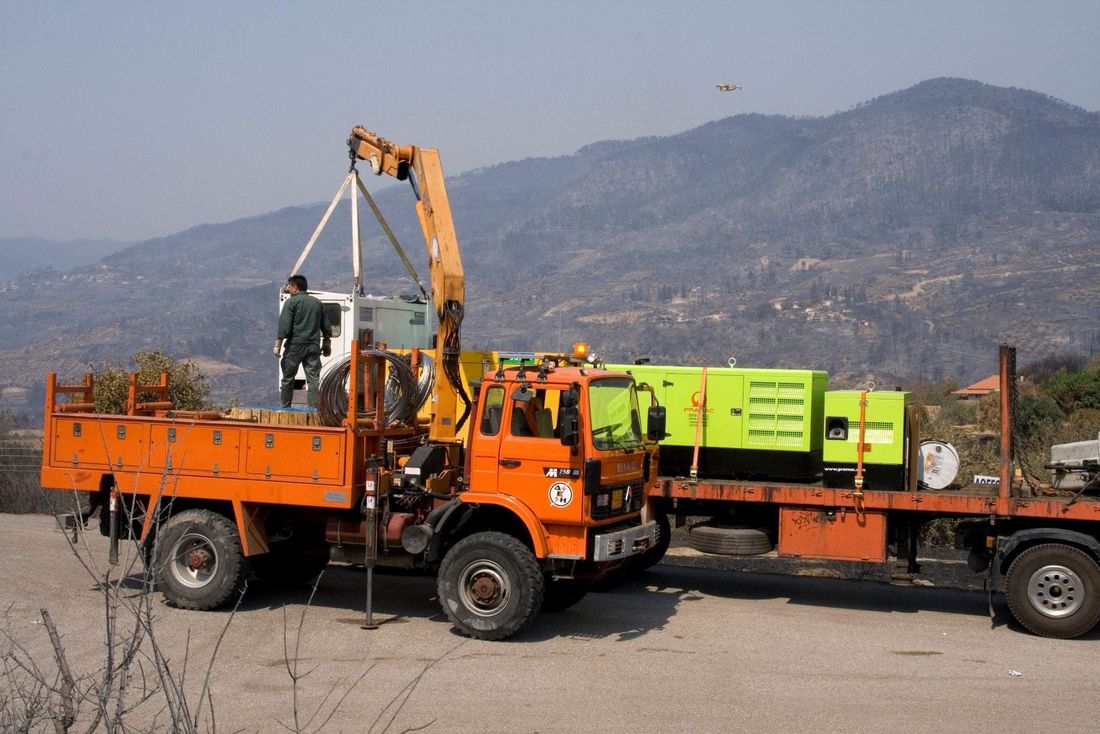
Figure 4 - Mobile diesel generators used for system restoration
(Source: Courtesy of Public Power Corporation, Greece)
Other examples of system operation with preventive security can be found in Australia where wildfires have great impacts on both transmission (Fig. 5) and distribution networks. For instance, following the bushfire on 19 December 2014, when three major 330 kV transmission lines close to Perth in Western Australia tripped in close succession due to flashover caused by smoke, some portions of the network may now be run under N-3 security to prevent risk of cascading in the presence of nearby bushfires. Severe bushfire events such as in 1983 (“Ash Wednesday”) and 2009 (“Black Saturday”) have also driven the evolution of sophisticated mitigation strategies in the state of Victoria. Besides frequent asset and vegetation easement inspection, a major noteworthy measure is the deployment of rapid earth fault current limiter (REFCL) devices, through which the phase-to-ground fault duration is reduced to milliseconds, so that and a fault current is usually less than 0.5 amps, decreasing the probability of starting a fire by up to 55%.
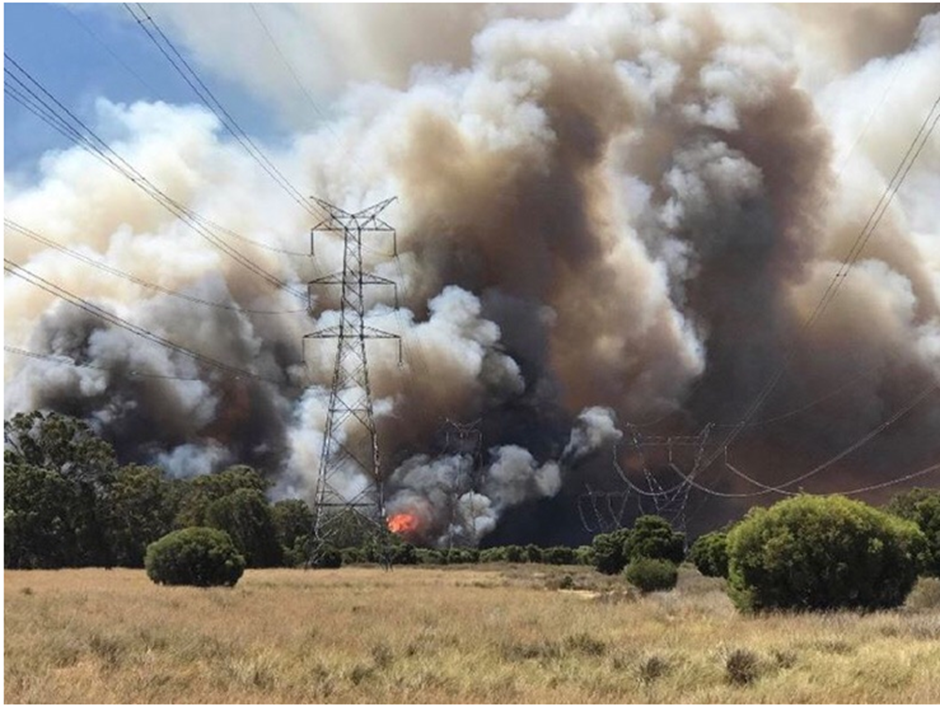
Figure 5 - Example of wildfires impacting multiple transmission corridors in Australia
(picture courtesy of Dean Sharafi; source: [3])
Overall, a combination of the measures described above is becoming increasingly important while longer and drier periods keep occurring in regions such as California, Greece and Australia, subject to high winds and extended droughts.
Conclusions
The frequency and intensity of wildfires are expected to increase and so the need to protect power systems against such events. In this vein, this paper provides an overview of measures related to infrastructure and operational resilience, including both a theoretical framework and real world examples. It emphasises the need to balance the portfolio solution among several options to make the system more robust, redundant, flexible and responsive, using a mixture of wires and non-wires innovative smart grid technologies. Asset management technologies and inspection, situational awareness and forecasting, advance protection and control, and advanced operational measures are key to complement expansion and hardening measures at the infrastructure level.
Two key lessons from our analysis, with policy implications, are (i) the need to upgrade grid standards and mandates to provide resilient solutions as there is a growing consensus that the actual state of affairs (regulation and market design) does not suffices; and (ii) the need to capitalise on the future smart grid world and the digitalization of energy systems, which provides unique opportunities for smarter management of the electricity system during wildfires and other extreme events.
Further reading
- CIGRE WG C4.47 Power System Resilience, “International Survey on Adoption of Resilience Within the Electricity Sector”, Symposium Aalborg, Denmark, June 2019
- R. Moreno et al., "Microgrids Against Wildfires: Distributed Energy Resources Enhance System Resilience," in IEEE Power and Energy Magazine, vol. 20, no. 1, pp. 78-89, Jan.-Feb. 2022, doi: 10.1109/MPE.2021.3122772.
- D. Sharafi et al., "Wildfires Down Under: Impacts and Mitigation Strategies for Australian Electricity Grids," in IEEE Power and Energy Magazine, vol. 20, no. 1, pp. 52-63, Jan.-Feb. 2022, doi: 10.1109/MPE.2021.3122732.
Thumbnail credit: Malachi Brooks on Unsplash